Tomado de: A
Late Proterozoic-Early Paleozoic magmatic cycle in Sierra de la Ventana,
Argentina, D.A. Gregori,, V.L. López , L.E. Grecco, Journal of South American Earth Sciences xx (0000) xxx–xxx
A Late Proterozoic–Early Paleozoic magmatic cycle
in Sierra de la Ventana, Argentina
Located in southeastern Buenos Aires province,
Argentina, Sierra de la Ventana is a 180 km long
sigmoidal mountain belt, composed of basement and
sedimentary cover (Fig. 1).
The basement consists of
Late Precambrian–Early Paleozoic deformed granites,
rhyolites, and andesites. The Paleozoic sedimentary
sequences can be divided into three groups.
Conglomerates
and quartzites compose the Curamalal (Ordovician–
Silurian) and Ventana (Silurian–Devonian) Groups.
The
Pillahuinco Group (Upper Carboniferous–Permian) is
composed of glacial deposits, black shales, and
sandstones. Deformational episodes occurred during the
Upper Devonian and Permian.
Keidel (1916) correlated the Sierra de la Ventana
sequences with the Karoo basin in South Africa, and Du
Toit (1937) integrated both areas in the Samfrau
geosyncline. Andreis (1964), Andreis et al. (1989), Borrello
(1962) and (1964), Buggisch (1987), Harrington (1947),
(1970), (1972) and (1980), In˜iguez Rodrı´guez and Andreis
(1971), Kilmurray (1968a,b) and (1975), Kilmurray et al.
(1985), Llambı´as and Prozzi (1975), Selle´s Martı´nez (1989),
Varela (1973) and (1978) and von Gosen et al. (1990),
(1991) and (2002), among others, carried out geological
studies in Sierra de la Ventana.
In addition, Grecco et al.
(1984) and (1999), Grecco and Gregori (1993), Varela
(1985), Rapela and Pankhurst (2002), and Rapela et al.
(2001) and (2003) studied its petrography, geochemistry,
and geochronology and reported preliminary geotectonic
interpretations of the igneous rocks.
In this paper, we present new petrographical and
geochemical data about the intrusive and volcanic rocks
of Sierra de la Ventana. These are integrated with regional
geological data to postulate the magmatic source of the
rocks and propose a model for the Late Proterozoic–Early
paleozoic tectonomagmatic evolution of this part of
Gondwana.
Igneous rocks crop out at La Mascota, La Ermita, Agua
Blanca, Pan de Azucar, Cerro del Corral, San Mario, and Cerro Colorado (Fig. 1). Most are exposed on the western
side of the Sierra de la Ventana due to tectonic transport to
the northeast by thrust faults (Cobbold et al., 1986).
The outcrops at Cerro Colorado, La Ermita, and Agua
Blanca are granitic and rhyolitic rocks overlain by
Quaternary sediments. Those at San Mario, Pan de Azucar,
and Cerro del Corral show tectonic contacts with the
Paleozoic sedimentary cover (Fig. 1).
According to field relationships in the San Mario, Pan de
Azucar, and Cerro del Corral granites, as well as in the
Cerro del Corral rhyolite and the Pan de Azucar andesite,
these rocks can be grouped into the Meyer suite.
Strong ductile deformation and a typical geochemical
signature, as reported subsequently, characterize this suite. The Cochenleufú suite consists of the Agua Blanca and
Cerro Colorado granites and the La Ermita rhyolite. They are
slightly deformed and characterized by a distinctive
magmatic fabric.
2.1. Meyer suite
2.1.1. Pan de Azucar
In Pan de Azucar (Fig. 2a), the greenschists, metaquartzites,
basic bodies, and mylonitized granites described by
Cuerda et al. (1975) underlie the Paleozoic sequence (Pan de
Azucar Formation). Schiller (1930) and Cucchi (1966) infer
a tectonic contact, whereas von Gosen et al. (1990) find no
evidence of intrusive contact. Cobbold et al. (1986) suggest a dextral overthrust to the northeast. Our interpretation,
based on the presence of ultramylonitic belts (3448/618SW)
in the granitic rocks, is a NE-vergent overthrust.
As shown in Fig. 2a, the eastern flank of the Cerro Pan de
Azucar consists of deformed plutonic and volcanic rocks.
Plutonic rocks exhibit isogranular to ultramylonitic textures.
The former are composed of quartz, twinned K-feldspar,
plagioclase (An26 – 33), and biotite. Plagioclase is replaced
by sericite, epidote, and calcite. In the QAP modal triangle
(Fig. 2a), these rocks plot in the synogranite and
monzogranite fields. In protoclastic and protomylonitic
varieties, quartz, plagioclase, and K-feldspar were disrupted
to produce porphyroclasts with patchy extinction and
fracturing. In the mylonitic granites, a fine-grained matrix
composed of quartz, biotite, sericite, and chlorite wraps
around rotated porphyroclasts of quartz, plagioclase, and
K-feldspar.
 |
The ultramylonitic granites display a few
strongly fractured porphyroclasts of K-feldspar in a
fine-grained matrix of quartz, muscovite, and chlorite with
minor calcite and biotite.
Basic and acidic volcanic rocks also are recognized in
this profile. Acidic rocks consist of a 1 m thick belt of
porphyritic rocks with 30% euhedral quartz, plagioclase
(An10 – 15), and K-feldspar fenocrysts in a groundmass of
micsrocrystalline quartz, alkaline feldspar, and fine micas.
Quartz shows embayment, whereas plagioclase contains
agglomerates. Its microscopic characteristics and QAP
diagram classification correspond with a rhyolite.
Basic volcanites appear as a 10 m thick, 90 m long body
with notable coarse fenocrysts of plagioclase. Kilmurray
(1968b) first described these rocks as diabases.
The groundmass displays a pilotaxitic texture with laths of
plagioclase and elongated crystals of tremolite, epidote, and
quartz. The plagioclase (An20 – 23) shows alteration to epidote and sericite. Oscillatory zoning and lamellar
twinning are common. On the basis of these characteristics,
the rocks are classified as andesites.
2.1.2. Cerro del corral
Cerro del Corral is located 100 m east of Pan de
Azucar, and together they constitute the core of a large
overturned anticline Ductile shear zones and belts of
mylonites and phyllonites on the eastern side of Cerro
del Corral are related to overthrusting on the Paleozoic
sequences. Protoclastic, protomylonitic, and mylonitic
granites appear on the western flank of the Cerro del
Corral (Fig. 2b). They are coarse- to medium-sized
grains with fractured quartz, K-feldspar, and twinned
plagioclase (An8–10). Secondary quartz, biotite, and
muscovite appear in veinlets or disseminated crystals.
On a QAP diagram, these rocks plot in the synogranite
and monzogranite fields.
Volcanic rocks display porphyritic, protomylonitic, and
mylonitic textures. Porphyritic varieties are composed of
20% quartz, alkali feldspar, and plagioclase phenocrysts.
Quartz appears as subhedral phenocrysts with embayment
and shadowy extinction. The alkali feldspars are euhedral
and exhibit simple twinning and flame-like pertites.
The scarce plagioclase phenocrysts (An10 – 12) have diffuse
lamellar twinning. The matrix consists of muscovite, biotite,
calcite, subhedral quartz, and plagioclase (An10). They are
petrographically classified as rhyolites. Micaceous-rich, coarse bands of quartz and alkali
feldspar compose the mylonitized rhyolites. The original
igneous quartz and alkali feldspar represent porphyroclasts
or augens surrounded by a deformed matrix.
2.1.3. Cerro San Mario
In San Mario, an E–W, elongated, granitic body (600 m
long, 150 m wide) is overthrust on conglomerates of the
Curamalal Group. The San Mario granite is a medium- to
coarse-grained biotite monzogranite with protoclastic to
protomylonitic textures (Fig. 2c). The mineralogy consists
of quartz, K-feldspar, plagioclase, and biotite. Plagioclase
ranges from An15 – 33 in synogranites to An26 – 40 in
monzogranites. Large alkali feldspar is usually perthitic
and shows shadowy, crosshatched twinning.
Several foliated belts cut the granite N–S (3538/668SW).
Minor aplite and pegmatite dikes crosscut the granites.
Delpino and Dimieri (1992) recognize two deformational
events. The first is evidenced by a regional NNW
schistosity, and NE shear planes represent the second.
2.2. Cochenleufú Suite
2.2.1. Agua blanca
The Agua Blanca granite occurs 10 km north of Pan de
Azucar It is a porphyritic to aplitic granite, composed of
microcline, anorthoclase, quartz, and plagioclase
Quartz appears as isolated phenocrysts, whereas plagioclase
(An0–5) is subhedral to euhedral. Biotite with strong
pleocroism and muscovite are scarce. Fluorite is present
as disseminated crystals or veinlets in the microcline
(Grecco, 1990). Most samples from the Agua Blanca
granite plot on the monzogranite field in a QAP diagram
(Fig. 2d). Dimieri et al. (1990) describe kink bands,
deformational twinning, and flexures in microcline and
biotite crystals, which suggests ductile deformation during
the solid-state stage (Simpson, 1985; Paterson et al., 1989).
2.2.2. La Ermita
La Ermita is located 10 km NNW of Agua Blanca It
consists of a N–S, 500 m long hill with no relationship to
the Paleozoic cover. These rocks display fluidal and
microporphyritic textures with quartz and K-feldspar
phenocrysts. Quartz is anhedral and presents undulose
extinction and parallel deformational bands. The groundmass
is completely recrystallized to fine-grained quartz.
Fluorite and iron oxides appear as disseminated crystals.
These rocks are classified as rhyolites.
2.2.3. Cerro colorado
Cerro Colorado is located 20 km W of Cerro San Mario
The predominant lithology is a medium to coarse granite
(Fig. 2d). Microcline, quartz, and plagioclase are the most
abundant minerals; biotite, muscovite, fluorite, zircon,
apatite, and magnetite are accessory minerals. Quartz
appears as small to medium clusters between the microcline
or as large phenocrysts. Microcline appears as large
(3–5 cm) euhedral to subhedral crystals. Plagioclase
(An6–10) is euhedral with crystals up to 10 mm long, often
twinned with albite and albite-carlsbad. Micaceous minerals
are pleochroic biotite and kink-banded muscovite flakes.
Fluorite appears as disseminated crystals in K-feldspar,
irregular veinlets, and small lenses.
The western side of Cerro Colorado contains a foliation
marked by the orientation of K-feldspar megacrysts
(3–5 cm long) and mica flakes. The K-feldspar megacrysts
are aligned in two trends: L1 (55–658/N300–1208) and L2
(55–658/N20–2008). Biotites display a 2898/448SW to
3408/358SW foliation. Biotite cumulates also follow this
foliation. Orientations of feldspar and micas form an angle
of 20–408 and display a S–C foliation. This fabric was
developed during a submagmatic stage (Miller and
Paterson, 1994; Tribe and D’Lemos, 1996). Dimieri et al.
(1990) describe similar features in the Agua Blanca granite.
3. Geochemistry systematics
Major, trace, and rare earth elements (REE) geochemical
data for both suites appear in Tables 1 and 2. X-ray
fluorescence was used to determine major, minor, and trace
elements: SiO2, TiO2, Al2O3, FeO, MnO, MgO, CaO, Na2O,
K2O, P2O5, Ba, Cl, Co, Cr, Cu, Ga, Nb, Pb, Rb, Sr, Th, U, V,
Y, Zn, and Zr. International geostandards, including AC–E,
MA–N, GS–N, GA, and GH granitoids, were used for
instrumental calibration. The analyses were carried out at
University of Barcelona. Instrumental neutron activation
analyses were carried out on 31 selected samples to
determine the REE, Ta, Hf, Sc, Cs, and Sn at ACTLABS.
3.1. Meyer suite
3.1.1. Major elements
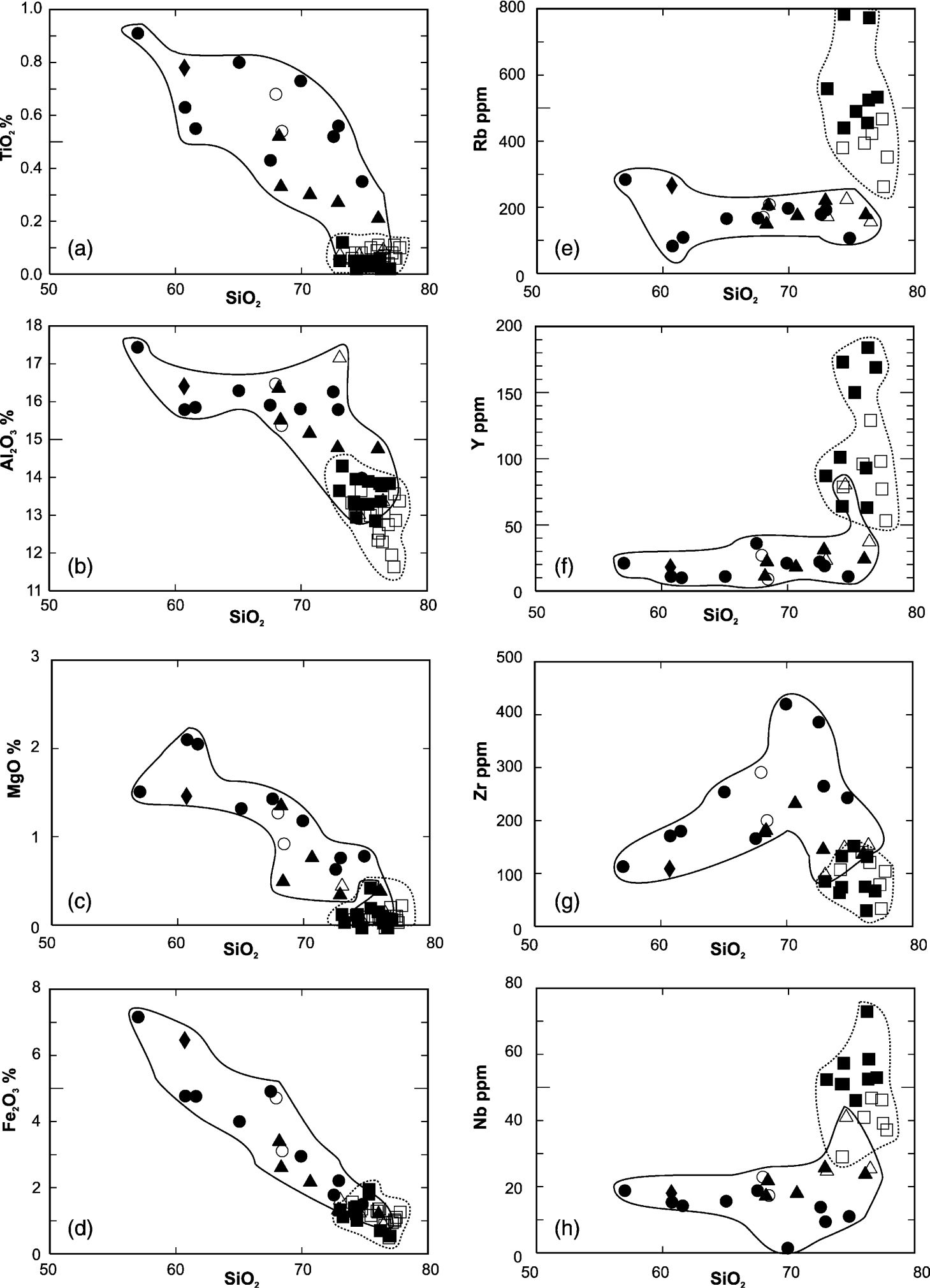 |
The granitoids of the Meyer suite are the most mafic
of the Sierra de la Ventana igneous rocks, ranging in
composition from monzonite to granite . Harker
diagrams of major elements display decreasing TiO2,
Al2O3, Fe2O3, MgO (Fig 3a–d), and CaO and increasing
K2O with increasing fractionation, thus implying a
comagmatic origin for these rocks. SiO2 concentrations
are between 57 and 77 wt% in the Pan de Azucar
granite, 67–76 wt% in the Cerro del Corral granite, and
68–76 wt% in San Mario. The granitoids are characterized
by high K2O (Cerro del Corral and Pan de Azucar:
6.29 wt%, San Mario: 5.28 wt%) and fall in the
shoshonitic and high-K calc-alkaline fields of Pecerrillo
and Taylor (1976) classification.
The AFM diagram (not shown) indicates a calc-alkaline
evolution, with A ranging from 55 to 85 and evolving from
the Pan de Azucar andesite to the Cerro del Corral rhyolite.
The granitic rocks occupy the transitional terms of this
series. All samples are subalkaline.
The ASI index ranges 0.83–2.3 for the Cerro Corral and
Pan de Azucar granites and 1.9–1.1 for San Mario; for the
Pan de Azucar andesite, it is 1.3, and for the Cerro del Corral
rhyolites, it ranges 1.0–3.0. This range plots in the
peraluminous field on Shand (1943) diagram (not shown).
The normative corundum reaches 8.9% in the Cerro del
Corral granite, 9.4% in Pan de Azucar, and 7.1% in San
Mario, though modal corundum was not recognized. On the
basis of their petrographical and geochemical characteristics,
the Cerro del Corral, Pan de Azucar, and San Mario
granites are interpreted as S-types. The Cerro de Corral
rhyolites plot in the peraluminous field of Shand (1943)
diagram and in the banakite and high-K rhyolite field of
Pecerrillo and Taylor (1976) classification.
3.1.2. Trace and REE
Granites of the Meyer suite display significant variations
in trace element contents (Table 1) Fractional crystallization
is well represented by the K/Rb ratio, which decreases from
401 to 184 in the Pan de Azucar granite, from 261 to 189 in
the Cerro del Corral granite, and from 252 to 197 in the San
Mario granite. With increasing fractionation, Ba, Sc, Mn,
and Zr decrease and Ga, Ta, Nb, and Y increase (Fig. 3e–h).
This indicates fractional crystallization of plagioclase and
K-feldspar in the melt, with enrichment of Rb and depletion
of Sr in later stages (Grecco et al., 1999).
The total REE reaches 539 ppm in Pan de Azucar,
195 ppm in Cerro del Corral, and 180 ppm in San Mario.
Chondrite-normalized spider diagrams of Pan de Azucar
(Fig. 4a) indicate strong enrichment of LREE. The LaN/LuN between 16 and 100 suggests that garnet remained as a
residual phase during the melting of the parental rocks.
The patterns for Cerro del Corral (Fig. 4b) also display
important LREE enrichments, though not as high as those of
Pan de Azucar. Their LaN/LuN relationships vary between
8.6 and 28.0 with small Eu anomalies, reflecting some
plagioclase fractionation. San Mario displays patterns
similar to those of Cerro del Corral, with LaN/LuN
relationships varying between 7.6 and 10.2 (Fig. 4c).
The Pan de Azucar andesite is classified by Winchester
and Floyd (1977) as andesite and alkaline basalt. The total
REE concentration reaches 83 ppm, with LaN/LuN of 5.35
and Eu/Eu* of 0.82 (Fig. 4d).
The Cerro del Corral rhyolite has a high concentration of
silica and alkalies, thus classifying it as a high-K subalkaline
(Leat et al., 1986). The total REE concentration reaches
164 ppm. Chondrite-normalized diagrams (Fig. 4b)
display severe negative Eu anomalies (Eu/Eu*:
0.097–0.42), which indicate that plagioclase was strongly
fractionated.
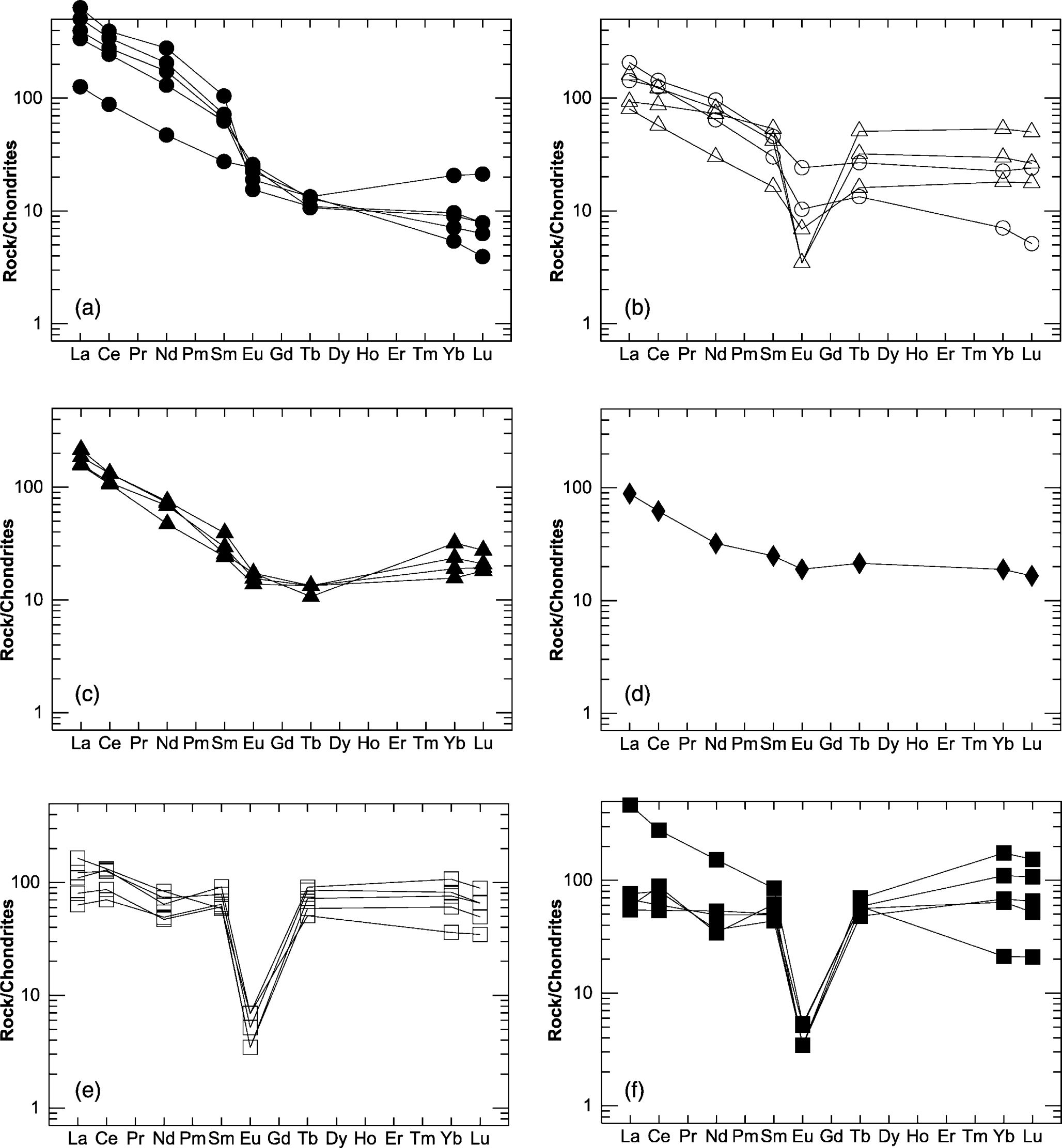 |
3.2. Cochenleufú suite
3.2.1. Major elements
The Agua Blanca and Cerro Colorado granites present
very restricted chemical compositions (Table 2) with SiO2 ¼ 73–77 wt%, Al2O3 ¼ 11–17 wt%, and K2O ¼
3:84–6:36 wt%: Fractional crystallization is not evident
from the Harker diagrams (Fig. 3a–d). The Agua Blanca
and Cerro Colorado granites are peraluminous and subalkaline
with 6.8 and 4.4 wt% corundum normative,
respectively.
3.2.2. Trace and REE
The Agua Blanca and Cerro Colorado granites present
restricted trace element concentrations, with Rb, Y, Nb,
and Ga concentrations higher than those of the Meyer
suite, whereas the Sr, Zr, and Ba concentrations are lower
(Fig. 3e–h). Logarithmic plots of Rb–Sr and Ba–Rb
indicate restricted fractional crystallization of plagioclase
and K-feldspar (Grecco et al., 1999). Total REE
concentrations are between 104 and 177 ppm in Cerro
Colorado and between 98 and 370 ppm in Agua Blanca.
The chondrite-normalized diagram for the Cochenleufu´
suite indicates enrichments of 60–150 times in Cerro
Colorado and 50–400 times in Agua Blanca (Fig. 4e and
f). Both bodies have strong negative Eu anomalies with
Eu/Eu* ¼ 0.049–0.094 in Agua Blanca and 0.051–0.127
in Cerro Colorado. These anomalies indicate that plagioclase
was severely fractionated from the original magma.
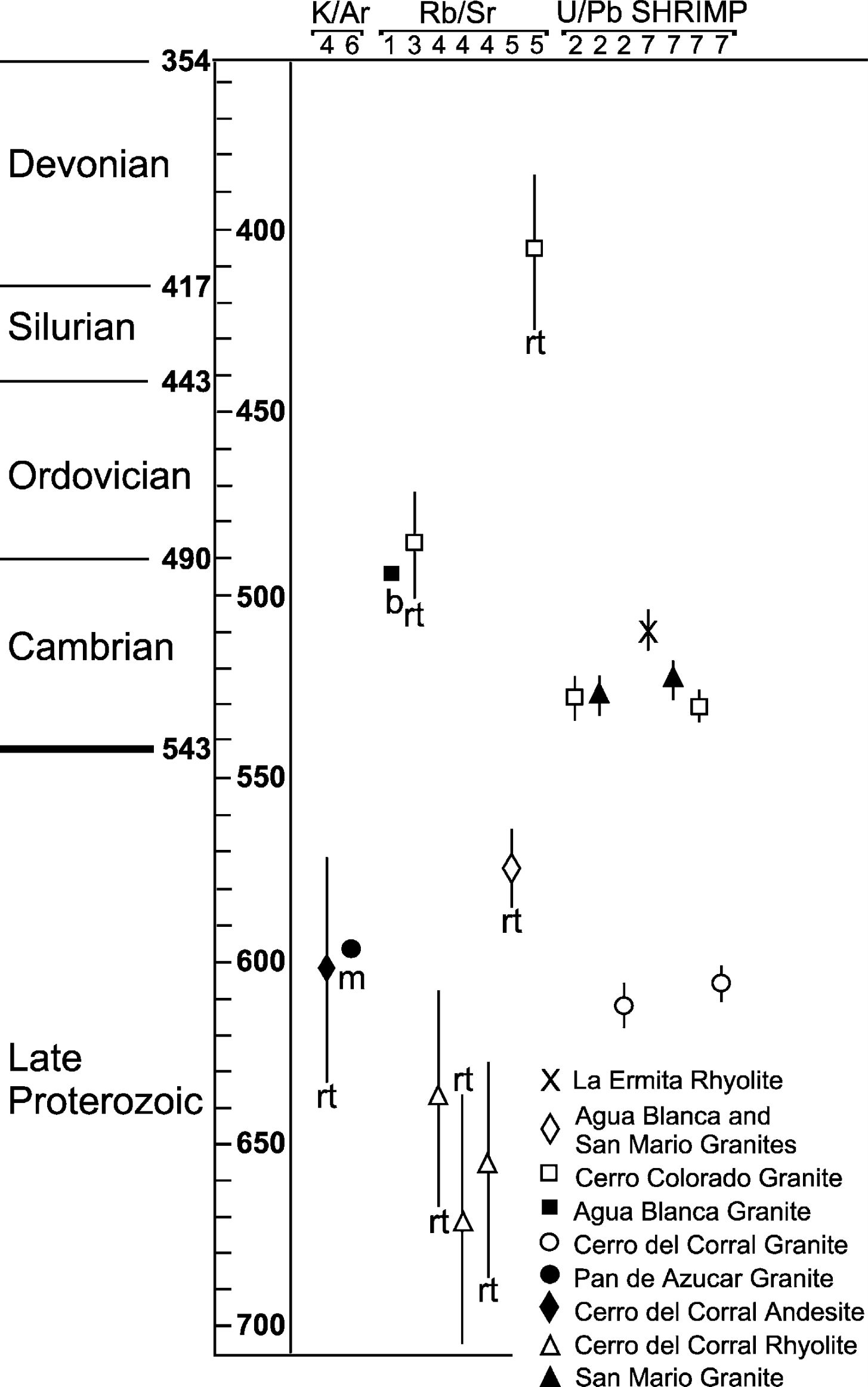 |
4. Previous geochronological studies Previous radiometric ages (Borrello and Venier, 1967; Cazeneuve, 1967; Cingolani and Varela, 1973; Varela
and Cingolani, 1975; Rapela et al., 2001, 2003) using K/Ar,
Rb/Sr, and U–Pb SHRIMP data have been determined
for the granitic and volcanic rocks of Sierra de la Ventana
(Fig. 5).
Late Proterozoic ages were obtained for the Cerro
del Corral rhyolite (671 ^ 35, 655 ^ 30, 638 ^ 30 Ma),
Cerro del Corral granite (612.3 ^ 5.3, 607 ^ 5.2 Ma), Pan
de Azucar andesite (603 ^ 30 Ma), and Pan de Azucar
granite (598 Ma).
Cingolani and Varela (1973) also constructed a Rb/Sr
isochron using samples from both the San Mario and Agua
Blanca granites to obtain an age of 574 ^ 10 Ma. Lower
Paleozoic ages were obtained for the Cerro Colorado granite
(487 ^ 15, 407 ^ 21, 529.6 ^ 3.3, 531.1 ^ 4.1 Ma), Agua
Blanca granite (492 Ma), San Mario granite (524.3 ^ 5.3,
526.5 ^ 5.5 Ma), and La Ermita rhyolite (509 ^ 5.3 Ma).
The age population indicates two magmatic cycles at
700–560 and 530–470 Ma, which are concordant with the
field-, petrographical-, and geochemical-based separation
into two suites. Discrepancies arise when the younger age of
the San Mario granite is compared with other members of
the Meyer suite. However, zircon cores with ages that vary
between 600 and 540 Ma and the Pb loss registered at
500 Ma suggest that the obtained age is not completely
reliable, especially considering the strong similarities in the
geochemistry, petrography, and deformation of the San
Mario, Pan de Azucar, and Cerro del Corral granites. On this
basis, the San Mario granite is included in the Meyer suite. Equivalent magmatic cycles have been recognized in
South Africa, where they constitute the Cape granite suite
(Scheepers, 1995; Scheepers and Poujol, 2002).
5. Tectonic discrimination
5.1. Meyer suite
Pearce et al. (1984) subdivide the granitoids, according
to their tectonic setting, into intrusion in ocean ridge,
volcanic arc, within plate, orogenic, and syncollisional
granites. In the Y þ Nb versus Rb diagram (Fig. 6a), samples plot dominantly in the volcanic arc field, whereas in
the Y versus Nb diagram, they plot in both the volcanic arc
and syncollisional fields (Fig. 6b).
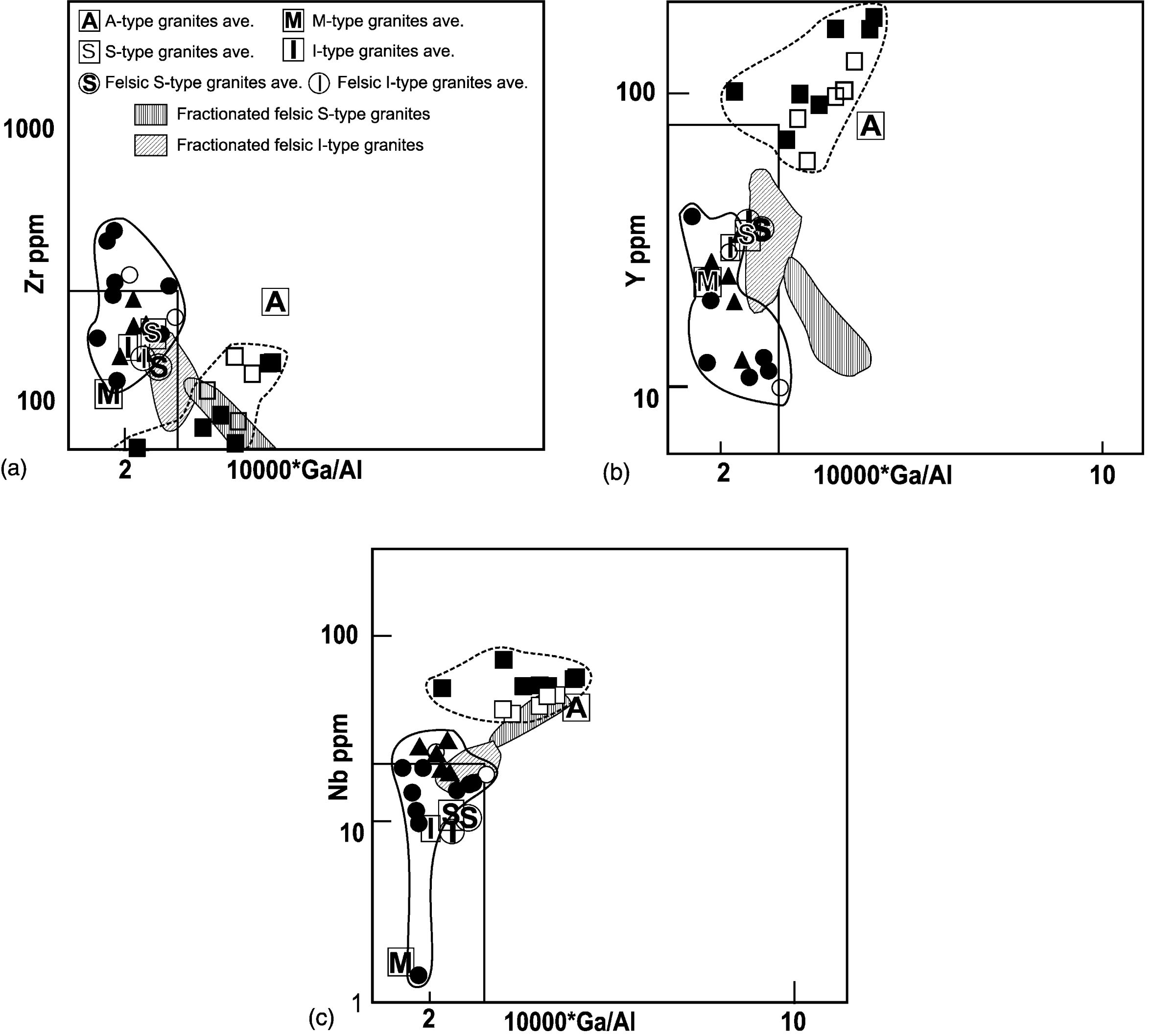 |
Whalen et al. (1987) discriminate between A-type
granites and most orogenic granitoids (M-, I-, and Stypes)
using the Ga/Al relationships and Y, Nb, and Zr
concentrations. In Whalen et al.’s (1987) discrimination
diagrams, the Meyer suite granites plot in the S- and I-type
granite fields (Fig. 7). These results indicate that the Meyer
suite consists of S-type granites, as defined by Chappell and
White (1974).
Harris et al. (1986) recognize four groups of granitic
rocks in the Himalayan, Alpine, and Hercynian belts: (1)
precollisional, calc-alkaline, dioritic to granodioritic plutons
associated with volcanic arcs; (2) syncollisional,
peraluminous leucogranites; (3) late- or postcollisional,
calc-alkaline, biotite-hornblende tonalite to granodioritic
plutons; and (4) postcollisional alkaline rocks.
In the
triangular diagram Rb/30-Hf–Ta*3 (Fig. 8a), samples plot
in the precollisional (volcanic arc) and the late- or
postcollisional fields, though a few appear in the withinplate
field. In the SiO2 wt% versus Rb/Zr diagram
(not shown), most samples plot in the VAG field. In the
Y–Nb–Ce and Y–Nb–3*Ga diagrams of Eby (1992), most
samples plot in the A1-type granite field (Fig. 9a and b).
Rocks with geochemical and petrologic characteristics
emplaced during a subduction-collision event have been
recognized in the Karakoram Axial batholith of northern
Pakistan (Baltoro plutonic unit, Hunza leucogranite;
Crawford and Windley, 1990), the Pan-African belt of the
Arabian shield (Jackson et al., 1984), the Homrit Waggat
complex of Egypt (Hassanen, 1997), and the Qinling belt of
China (Mattauer et al., 1985).
Through plotting in TiO2–MnO*10-P2O5*10, Ti/100-
Zr–Y*3, and Th/Yb–Ta/Yb discriminant diagrams (Pearce
and Cann, 1973; Pearce, 1982; Mullen, 1983), the Pan de
Azucar andesite is classified as a calc-alkaline basalt that
erupted at active continental margins (not shown).
5.2. Cochenleufu suite
Samples from the Cochenleufu´ suite plot in the withinplate
granite field (Fig. 6) of Pearce et al. (1984) diagrams.
They also fall in the A-type granite field in trace elements
versus 10000 Ga/Al discrimination diagrams (Fig. 7).
This result agrees with the A-type granite characteristics
of these rocks, namely, the high SiO2, Na2O þ K2O, Ga/Al,
Zr, Nb, Ga, Y, and Ce and low CaO. In the Rb/30-Hf–Ta*3 diagram (Harris et al., 1986), the
samples plot in the syn- to postcollisional fields (Fig. 8b); in
the SiO2 wt% versus Rb/Zr diagram (not shown), they are
classified in the syncollisional field.
Finally, in Eby (1992) Y–Nb–Ce and Y–Nb-3*Ga
diagrams, most of the samples plot in the continental–
continental collisional to postcollisional field (Fig. 9a and
b). Examples include the Gabo and Mumbulla suites in
the Lachland fold belt, Australia (Collins et al., 1982),
and the Topsails complex of Newfoundland (Whalen
et al., 1987).
6. Discussion
In Sierra de la Ventana, the deficiency of igneous rock
outcrops, as well as the lack of field relationships with
host rocks, makes it difficult to establish its tectonic setting. However, the correlation with the African
granitic suites enables us to clarify the evolution of
accretional events in Sierra de la Ventana. Two igneous
suites are differentiated in Sierra de la Ventana. The
most basic is the Meyer suite (700–570 Ma), which
displays a calc-alkaline evolution related to volcanic arc
and postcollisional settings.
 |
In the Saldania belt of South Africa, sedimentary and
volcanic rocks deposited in the Boland terrane (Malmesbury
Group) attest to ocean floor spreading in response to the
breakup of Rodinia and the progressive opening of the
proto-Atlantic (Adamastor Ocean) during 780–750 Ma
(Rozendaal et al., 1999). Rocks of this age, which represent
this extensional event, had not been recognized in Sierra de
la Ventana, though they were found in Sierra de Tandil.
A reversal of the spreading caused subduction and the
closure of the Adamastor Ocean (600–570 Ma).
The presence of a suture zone at the Swartland-Boland
terrane boundary supports either oblique collision or strikeslip
transpressional tectonics without the development of a
proper collision orogen. The Cape granite suite consists of a
first and second phase of intrusion (600–520 Ma), with
olivine gabbros, gabbros, diorites, and granodiorites related
to subduction along an immature magmatic arc (Scheepers,
1995). S-type granitic rocks that intruded during this phase
have late orogenic to postorogenic signatures. In Sierra de la
Ventana, the Meyer suite represents this subductioncollision
event.
The Cochenleufu´ suite (540–470 Ma) is considered an
A-type association and plots in the within-plate, syn- to
postcollisional fields in several discrimination diagrams.
According to Eby (1992) classification, these rocks belong
to the A2 granitoids, which generally occur as final plutonic
events during postorogenic extension in collisional belts
(Crawford and Windley, 1990; Bonin, 1990).
In the Cape granite suite, the final phase of intrusion
(520–500 Ma) is represented by A-type granitoids (Scheepers,
1995), which are considered anorogenic alkaline
granites. The granites are related to the pressure release that
occurs during a strike-slip regime, with associated uplift and
extension after collision. Equivalent postcollisional
granites, intruded during an oblique N–S collision of the
Kalahari and Congo cratons, have been recognized in the
central Damaran orogenic belt, Namibia (Gresse and
Scheepers, 1993). They are related to the closure of the
Adamastor Ocean.
The Swartland and Tygerberg terranes (Saldania belt),
accreted in a transpressive regime to the Boland terrane
(600–630 Ma), are potential candidates for the Sierra de la
Ventana suites’ emplacement.
Similar structural styles, magmatism, and tectonic events
have been recognized in the Saldania, Ross, and Delamarian
orogens, indicating a common history (Rozendaal et al.,
1999).
The Ross event, responsible for major magmatism in
the Transantarctic Mountains, is represented partially by the
granitic Harbour intrusive complex (Gunn and Warren,
Fig. 9. (a, c) Y–Nb–Ce diagram (Eby, 1992) and (b, d) Y–Nb-3*Ga diagram for the Meyer and Cochenleufu´ suites.
1962), which was emplaced in a calc-alkaline arc. The Mid-
Proterozoic-Late Cambrian history of the Transantarctic
Mountains has been interpreted as a cycle of extension,
continental rifting, transpression, and subduction (Moyes
et al., 1993).
7. Conclusions
In Sierra de la Ventana, two magmatic suites are
differentiated. The older Meyer suite (700–570 Ma) displays
a calc-alkaline evolution related to a volcanic arc and
postcollisional setting. The younger Cochenleufu´ suite
(540–470 Ma) displays an A-type signature related to
the final plutonic events during postorogenic extension in
the collisional belts.
The remarkable similarities observed between the suites
of Sierra de la Ventana and the Saldania belt enables a
positive correlation. In both cases, primitive volcanic arc or
collisional orogens are recognized. Moreover, continuous
shearing between the Swartland and Tygerberg terranes was
a potential trigger for the emplacement of both suites.